Longevity
Nick Schaum, PhD, Fellow
May 2024
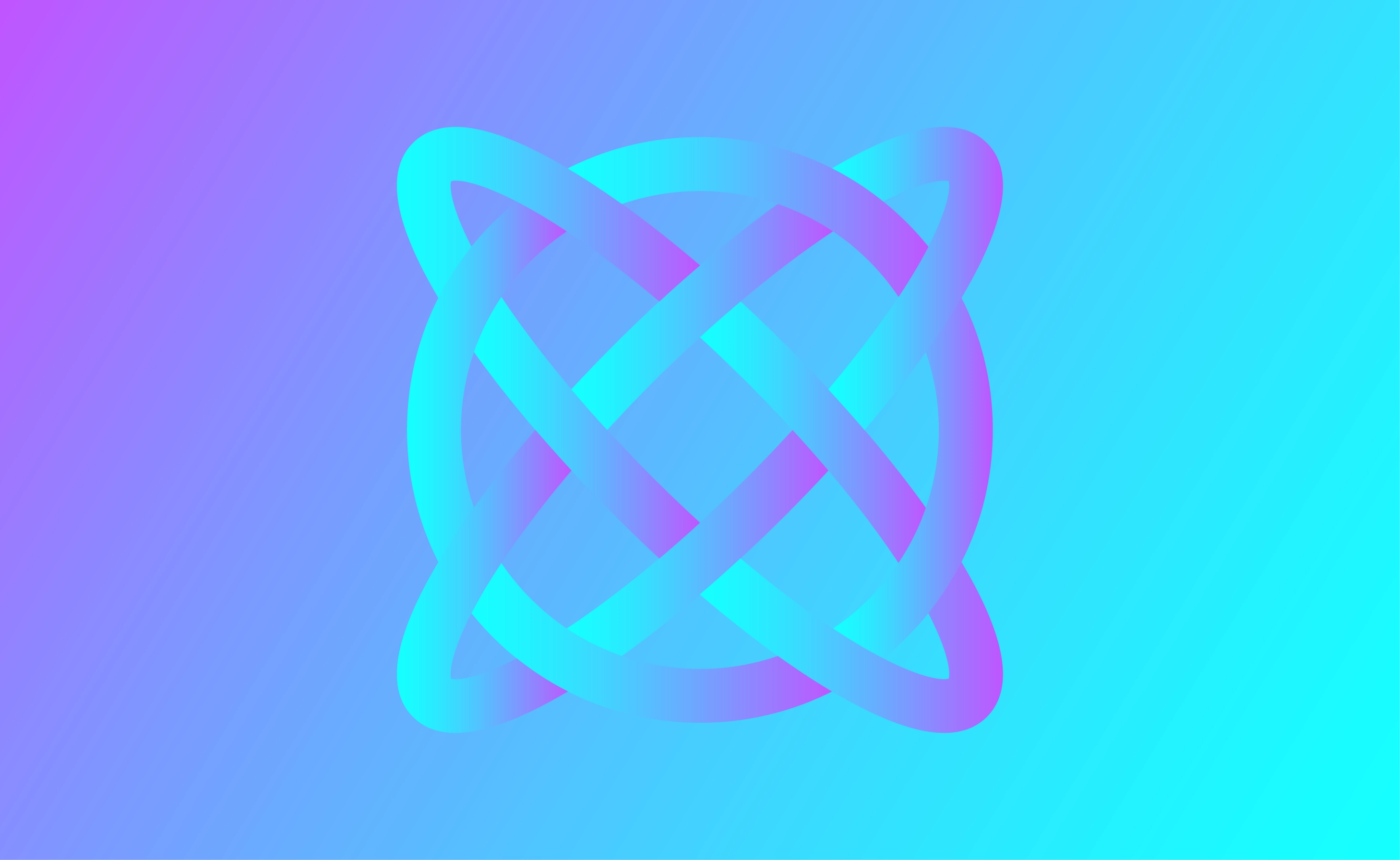
Aging Biotech: Moving Beyond the Hallmarks
The goal of scientists researching the biology of aging is often misunderstood to be extending lifespan at all costs, even if that means living longer in a decrepit and frail state. The reality is that no one, especially those of us studying aging, desires this. Who would want to live like that? The goal of aging research is exactly the same as that of modern medicine: keep us as healthy as possible for as long as possible. The key difference is the means by which this is achieved. Modern medicine is almost entirely reactive: diseases are tackled only once symptoms arise, often after the disease has progressed so much that reversing it is difficult or impossible. Frequently, symptoms aren’t even evident: think of all the cancers, clogged arteries, and dying neurons that progress over years and decades without detection at all. I don’t know about you, but I would rather not get these diseases in the first place! Can’t something be done to prevent them? To prevent the progressive decline of every single part of our bodies? To avoid the need for chemotherapy, triple bypass surgery, and weekly dialysis?
This is precisely why aging researchers study aging. We recognize that there are underlying biological mechanisms at play that progressively change our biochemistry — how our cells, tissues, and organs function and interact with each other. These mechanisms are the root of nearly all the diseases we are likely to experience in our lives, those we are likely to suffer from for years and often decades: heart disease, cancer, diabetes, Alzheimer’s. Arguably worse are the often unseen changes — arthritis, incontinence, and the many chronic aches and pains those of us still young cannot possibly appreciate.
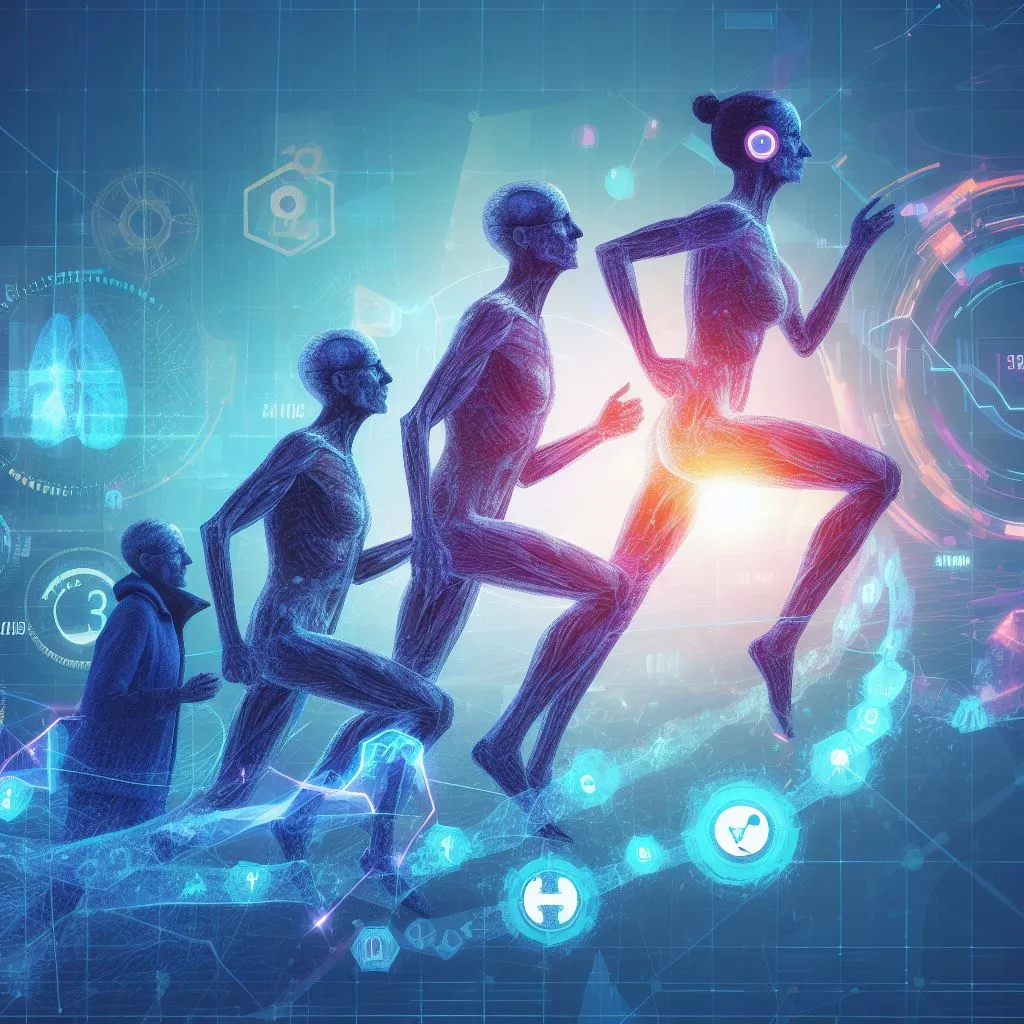
If we understood the underlying causes — the underlying biology of aging — shouldn’t it be possible to prevent these maladies from emerging in the first place? Scientists have been trying for decades to unravel it (and individuals have searched for a solution over the millennia), but the biology of aging is extremely complex. Halting or reversing the molecular process of aging is perhaps the most daunting challenge ever attempted. But isn’t that all the more reason to try? We may not need to “cure aging” entirely to elicit profound good. Even incremental progress could have profound implications — literally, every person in the world could benefit. If you’re not old enough to have overtly experienced aging yourself, you certainly know someone who has.
So what progress has been made? What have aging researchers actually accomplished, and what is left to be done? Well, most progress is in model organisms — nonhuman species used for biomedical research — often yeast, worms, fruit flies, and mice. We know much more about how these organisms age than humans do, because unlike with humans, we can study these organisms in controlled settings, with genetic tools, using invasive assays to understand vital, internal organs. Overall, there is far more unknown about aging than is known, although we do have a solid grasp of the major categories of what goes wrong: the hallmarks of aging. This includes things like damage to DNA, the dysfunction of mitochondria that convert nutrients to fuel for our cells, and alterations to communication between cells. But what is not often depicted is the extremely complex web of interactions between these hallmarks, making cause and effect nearly impossible to disentangle. Which hallmarks arise first? In which part of the body? In which cells? If you fix one hallmark, will others improve? Or do you need to fix several simultaneously? Many, but not all, of these processes also occur in humans, or they occur but in different ways. And humans also exhibit more unique aging characteristics not always evident in other species: menopause, Alzheimer’s, and atherosclerosis come to mind, to name a few.
Reassuringly, scientists have actually made substantial progress getting model organisms to live longer, despite this incomplete picture. We’ve made yeast and worms live several-fold longer than normal, and though lifespan is much harder to increase the closer (evolutionarily) you get to humans, dozens of methods (drugs, treatments, genetic models) have extended mouse lifespan anywhere from 5% to 50% (non-genetic methods like drugs are more often less than 10%, if that). Unfortunately, we have no evidence that any of these work to extend lifespan in humans (yet!). The age-old advice of eat well and exercise is about as good as it gets, though the emergence of diabetes drugs like semaglutide (Wegovy/Ozempic) — with expanded applications for weight loss and heart disease — could have profound societal implications for healthspan (the concept of increasing the healthy years of life — often, but not always, this goes hand in hand with increased lifespan). And many researchers would argue that we should immediately start clinical trials based on a particular set of results seen across all of the model organisms listed above: the extension of both lifespan and healthspan by dampening a molecular signaling cascade known as the nutrient-sensing pathway.
The nutrient-sensing pathway is an example of a conserved pathway. That is, it is so fundamental to cellular functioning that it is found across nearly all eukaryotes (animals, plants, fungi). Though there are specific differences resulting from evolution, yeast, and the cells of worms, flies, mice, and humans all use this pathway to detect the presence of nutrients and respond appropriately. When nutrients are abundant, cells grow and divide. When scarce, cells go into protection mode, recycling their own components to provide the energy needed to survive until nutrients are present again. This recycling, or “self-eating”, is called autophagy, and is especially useful because it promotes the degradation of damaged and dysfunctional cellular components. This recycling is thought to be a core mechanism behind the benefits of caloric restriction and fasting. Dampen that signaling, stress your cells a bit (but not too much: see hormesis), and the end result is so beneficial that your body remains healthier for significantly longer.
Known to increase lifespan for over half a century, nutrient sensing has been perhaps the single largest topic within aging research. As this molecular pathway has been elucidated over the years, more and more therapies and drugs have been developed that attempt to manipulate it at various stages to improve health. In relatively recent history, a drug called rapamycin was shown to increase the lifespan of mice even if not started until old age. This was a monumental breakthrough in aging research because prior to that, it was largely believed that to increase lifespan, one must intervene in the aging process early. Since this time, rapamycin has grown to become the single most robust method of increasing longevity across organisms, with some members of the research community arguing it should be put into large-scale clinical trials right away (it is already FDA-approved at high doses as an immunosuppressant and cancer treatment). In this vein, academic labs and companies like Aeovian Pharmaceuticals, Beiwe Health, and Tornado Therapeutics, continue to work on rapamycin, rapalogs (variants of rapamycin), and other molecules with similar molecular targets. Others, like Trivium Vet and the Dog Aging Project, are taking this treatment to our pets, hoping that it can act as a stepping stone toward applications for humans.
But nutrient sensing is far from the only aging hallmark under investigation. In the last decade, senescent cell elimination has gained traction. A senescent cell is one that has ceased dividing after undergoing damage, typically DNA damage that could make the cell cancerous. This is a good thing, and cellular senescence is critical to preventing cancer (it may also aid wound repair, and it is essential for proper embryogenesis). The problem with senescence arises when senescent cells persist. Instead of being cleared by the immune system, they stick around, spewing pro-inflammatory cytokines and matrix-degrading enzymes into the surrounding tissue. With age, more and more senescent cells accumulate, and early efforts focused on dampening the secretion of these deleterious factors. But, once studies in mice genetically engineered to eliminate these cells showed longer lifespans, the race to develop senolytics — drugs that specifically kill senescent cells but leave healthy cells untouched — was on. One of the earlier players was Unity Biotechnology, which has advanced several candidates to the clinic, but now over a dozen companies are pursuing senescent cell elimination, either using drugs or, like Arda Therapeutics, by taking advantage of the immune system.
The remaining aging hallmarks are similarly under investigation, as the aging biotech space has exploded over the last decade. Now, over 200 companies directly state that they work on aging or longevity, and hundreds more are working on relevant tools, pathways, or indications. The field expanded so quickly from just a handful of companies that several individuals have compiled databases to keep track, including the Longevity Biotech Landscape, the Longevity Industry Database, and AgingBiotech.info. The hallmarks are wonderful for understanding the categories of problems that emerge with age, but they were never meant to comprehensively capture all features of aging, and notably, more and more biotechs are not targeting any hallmark per se. Some are starting from first principles, aiming to uncover new aspects of aging or new interventions that increase lifespan (you would be hard-pressed to find an intervention that increases lifespan without also increasing healthspan, by the way). The newly formed Ora Biomedical plans to screen one million compounds in worms to see which extend lifespan the most, and a similar approach was taken by Longevica in mice a decade ago. EPITERNA is screening approved compounds for lifespan and healthspan effects in yeast, worms, flies, fish, and mice. Gordian Biotechnology has a sophisticated discovery platform that, instead of using cells (or worms, etc.) in a dish for screening, they use cells in a live mouse, using genetic tools to test hundreds of interventions simultaneously in a diseased organ in its natural setting.
Other companies are similarly thinking outside the aging hallmarks box, developing technologies that reverse multiple characteristics of aging simultaneously. One of these approaches is partial reprogramming, a method that turns cells back toward a more stem cell-like state, without actually converting them back to stem cells. The breakthrough methods for reprogramming were published less than 20 years ago, and those results spurred a new era not only for aging research but for all biomedical sciences. Next time, we’ll delve into this revolution and what it means for aging, and then we’ll visit the concept of “replacement” — the idea of removing old cells, tissues, and organs, and replacing them with young ones. Though this sounds like science fiction, the technology has developed more than you may think, and many scientists believe this may be our best chance to really move the needle toward adding a significant number of healthy years to our lives.
Reprogramming: Rejuvenation Nature’s Way
While nutrient sensing has dominated research for decades, and eliminating senescent cells took off around a decade ago, the latest excitement revolves around something called reprogramming.
Even if you haven’t come across reprogramming, you are already familiar with its core feature: resetting cellular age to zero. We experience it with every new generation: your body’s cells are direct descendants of your parents’ cells, which were probably a few decades old at conception. Extend that back through the generations and it becomes clear that the cells that make up you go back millions — well, actually billions — of years. There is a continuous line of descent from the very first cells to those forming every part of you. Famines, floods, mass extinctions — your cells survived them all. They are, in this sense, immortal. So why aren’t we immortal? Why do our bodies grow old and die, yet we can propagate our cells in perpetuity simply by having children?
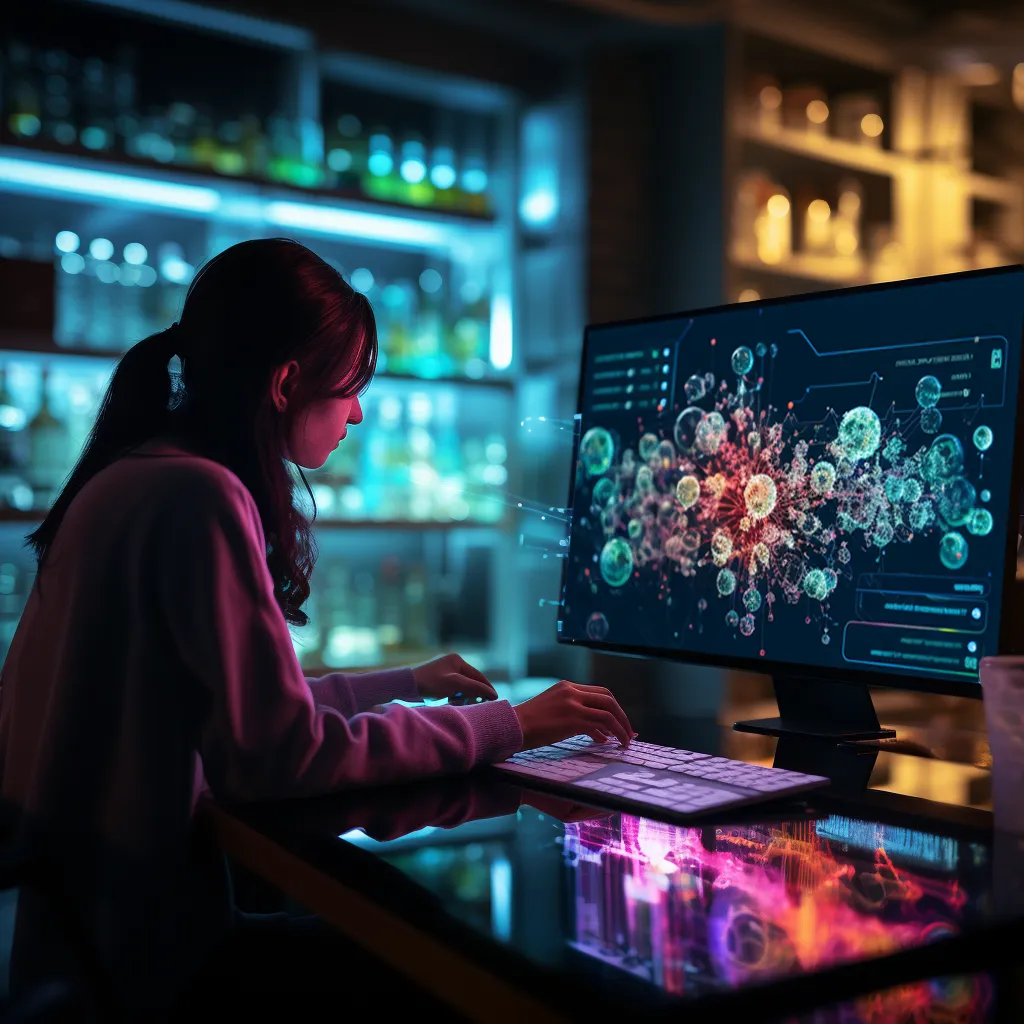
This is the crux of two concepts: the Immortal Germ Line, and the Disposable Soma Theory. Our germ (reproductive) cells persist indefinitely, but our bodies are mere vessels. Our germ cells don’t care what happens to “us.” They only care about being reproduced — copied widely and perpetually. Replication, duplication, expansion: these are the fundamental concepts of evolution, driven by our Selfish Genes. Whatever virus, or bacterium, or animal is best at making more of itself wins. At the level of evolution, that’s all life is: a replication competition.
So if the cells of our bodies must succumb to aging and death, how do reproductive cells survive forever? If they don’t age, or if they de-age, can we apply these rejuvenative molecular processes of reproduction outside the germ line? Scientists have searched for answers for decades. Some of the first real progress came with a technique called somatic cell nuclear transfer (SCNT). If you don’t recognize the name, you probably know its application: cloning. As in Dolly, the sheep. SCNT involves collecting the nucleus containing all the genetic, inherited information (the DNA) from a somatic cell (a non-reproductive cell, like the mammary gland cell in Dolly’s case) of the adult donor you want to clone. This nucleus is then physically transferred to an egg cell (oocyte) with its nucleus removed. After implanting the egg in a surrogate mother, it divides and grows into an exact genetic replica of the donor: a clone. Despite Dolly’s premature death, it now appears that cloned animals age no differently from normal animals. Old DNA can indeed create an entirely new, “day 0” organism. This perhaps marked the first hint that aging, at least at the DNA level, is not inevitable. But this wasn’t actually the major finding at the time. Instead, the core concept centered around the loss of cellular identity during SCNT. The DNA, originally specialized for mammary gland cell function, underwent a “reset.” It regained the ability to create every cell type in the mature body, just like an embryonic stem cell. In other words, mammary gland DNA was reprogrammed into an embryonic state.
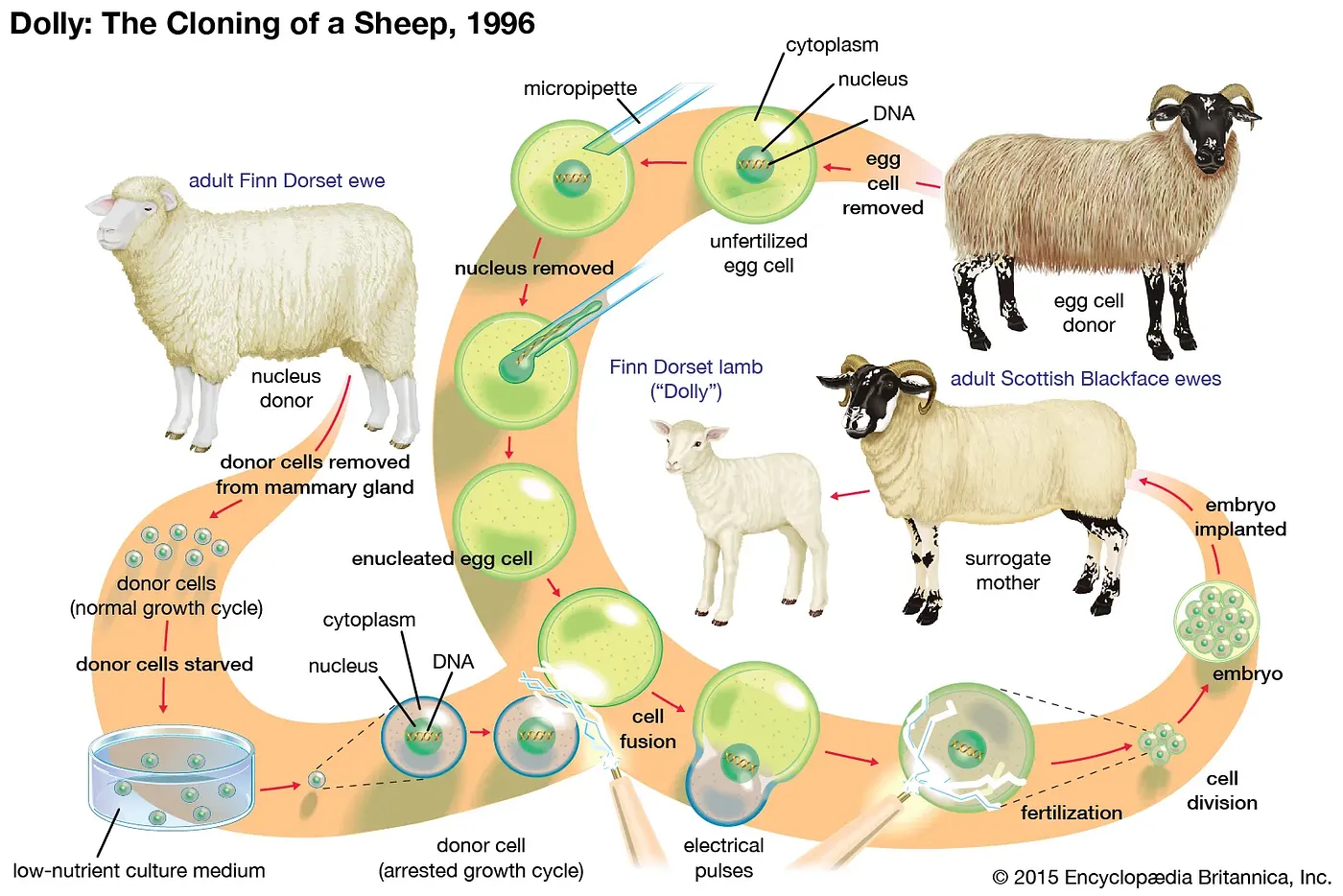
Source: Somatic Cell Nuclear Transfer
To grasp the implications, we need a little biology. Firstly, remember that every cell in your body harbors the exact same DNA. Starting from the one, single cell formed upon conception, this DNA is copied repeatedly and exactly, ultimately inhabiting the trillions of cells of your body. Yet, despite this uniform genetic blueprint, our cells are not identical. Far from it, they display remarkable diversity. How then do cells with identical instructions perform such different functions?
It boils down to which instructions are read, and which are not. While no perfect analogy exists, envision your DNA as an aggregation of 20,000 instruction manuals (in genetic terms, each manual is called a gene). But, rather than explicitly guiding the construction of a house (i.e. a cell), these manuals dictate the creation of basic building blocks and tools that go into creating and maintaining the house: the nails, screws, bricks, mortar, hammers, drills, etc. There are many building blocks and tools all cells require to perform basic functions, and all cells read this same core set of instructions (these are called housekeeping genes). But the adult body is composed of specialized cells, those that perform specific and distinct functions. What makes these cells different is that they read a different set of instructions. Some are turned off, others on. With 20,000 instructions, the potential combinations give rise to a vast array of cell types: muscle cells that contract, immune cells that kill bacteria, neurons that transmit electricity, pancreatic beta-cells that secrete insulin. Each cell type has a specific, defined subset of the 20,000 genes turned on, and the rest are off. This set is what defines the cell’s function, and thus its identity.
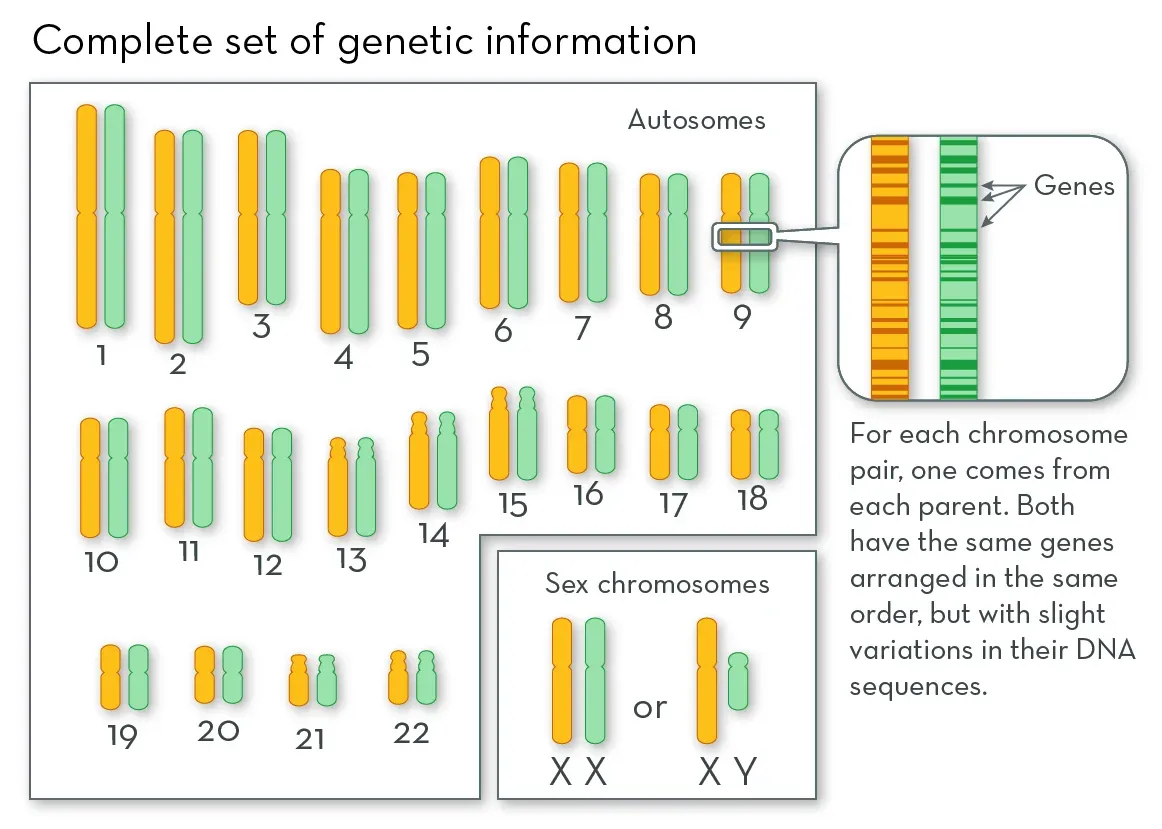
Source: What are Chromosomes?, the Genetic Science Learning Center, University of Utah Health Sciences
During reprogramming, when converting Dolly’s mammary gland cell DNA into the DNA of an embryonic stem cell, the set of genes that are on versus off is changed. Mammary gland-specific genes are turned off, while other genes are turned on. Although numerous complex mechanisms regulate gene activation, one extensively studied process is DNA methylation, in which a small chemical modification is added to the DNA. This methyl modification is typically repressive — that is, it blocks access to the on-switch of each gene. Mature cells, like adult mammary gland cells, exhibit highly methylated DNA — on-switches to many genes are blocked leaving only housekeeping genes and mammary gland cell-specific genes on. During reprogramming to an embryonic cell, similar to what occurs after fertilization during early embryo development, DNA undergoes extensive demethylation. The repressive chemical modifications are removed, priming the cell with the ability to activate almost any of the 20,000+ genes. This demethylation, and other associated molecular changes, is thought to underpin both the loss of cell identity (conversion to an embryonic stem cell) and the rejuvenation (resetting to age zero).
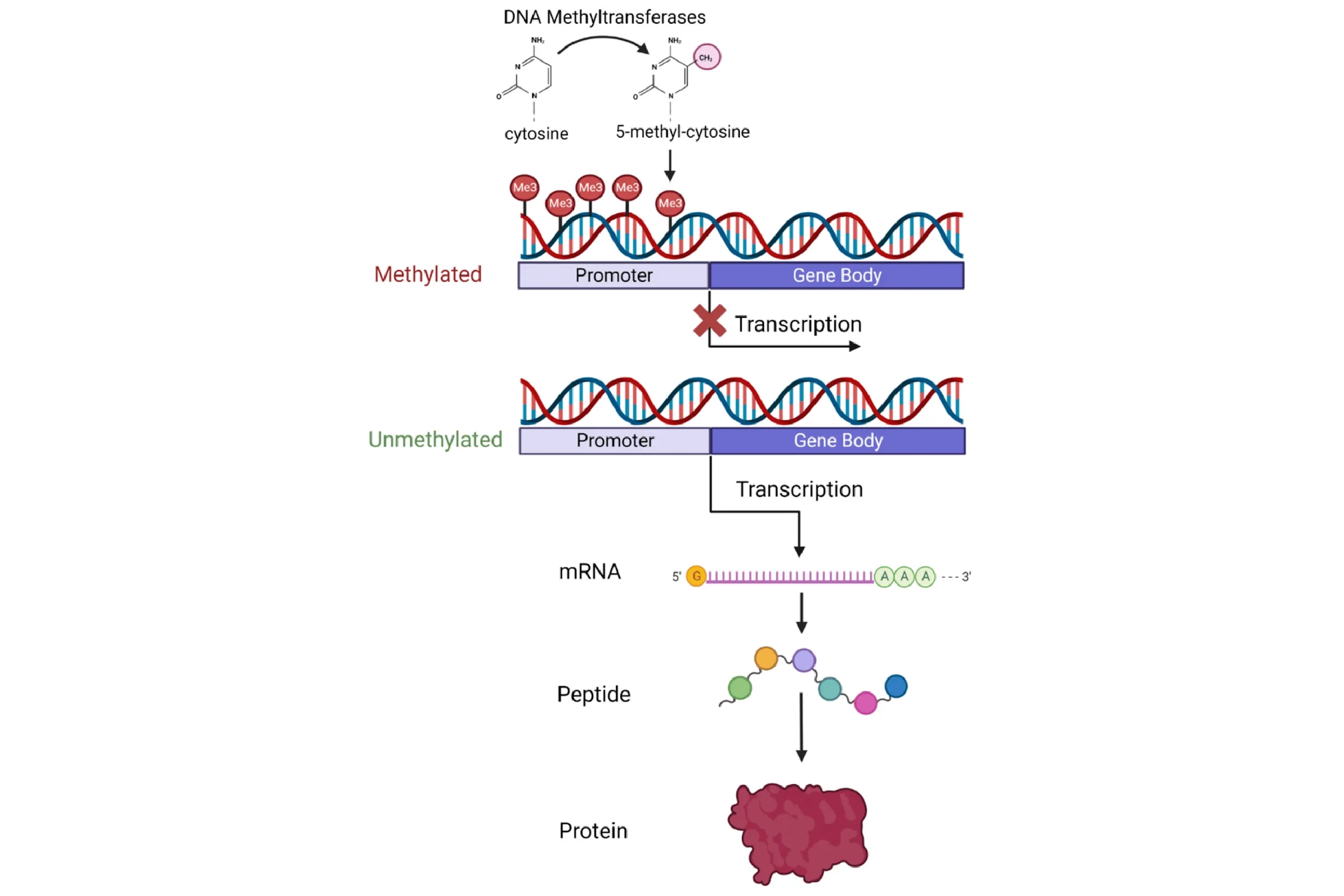
But why exactly does transferring a mature nucleus into an egg cell reprogram the DNA? What is inside this oocyte that triggers this process? A clue came in the early 2000s with the discovery that reprogramming also occurs when a mature cell fuses with an embryonic stem cell (ESC). This suggested that something inside the ESC spurs reprogramming, similar to the oocyte. The major breakthrough — and one that won a Nobel Prize — came in 2006, after researchers discovered four specific genes (abbreviated “OSKM”, also known as the “Yamanaka factors”) that, when activated simultaneously in a fully specialized cell, could reprogram it into an embryonic-like state capable of forming any specialized cell type — a state termed pluripotent. This discovery of induced pluripotent stem cells (iPSCs) marked a new era in biomedical research. With iPSCs came the promise of creating any cell type at will, opening endless possibilities for new treatments — a feat possible before only with ethically fraught and sparsely available embryonic stem cells. Using a patient’s own skin cells, for example, one could conceivably convert them into dopaminergic neurons and transplant them into Parkinson’s patients, all without the risk of immune rejection.
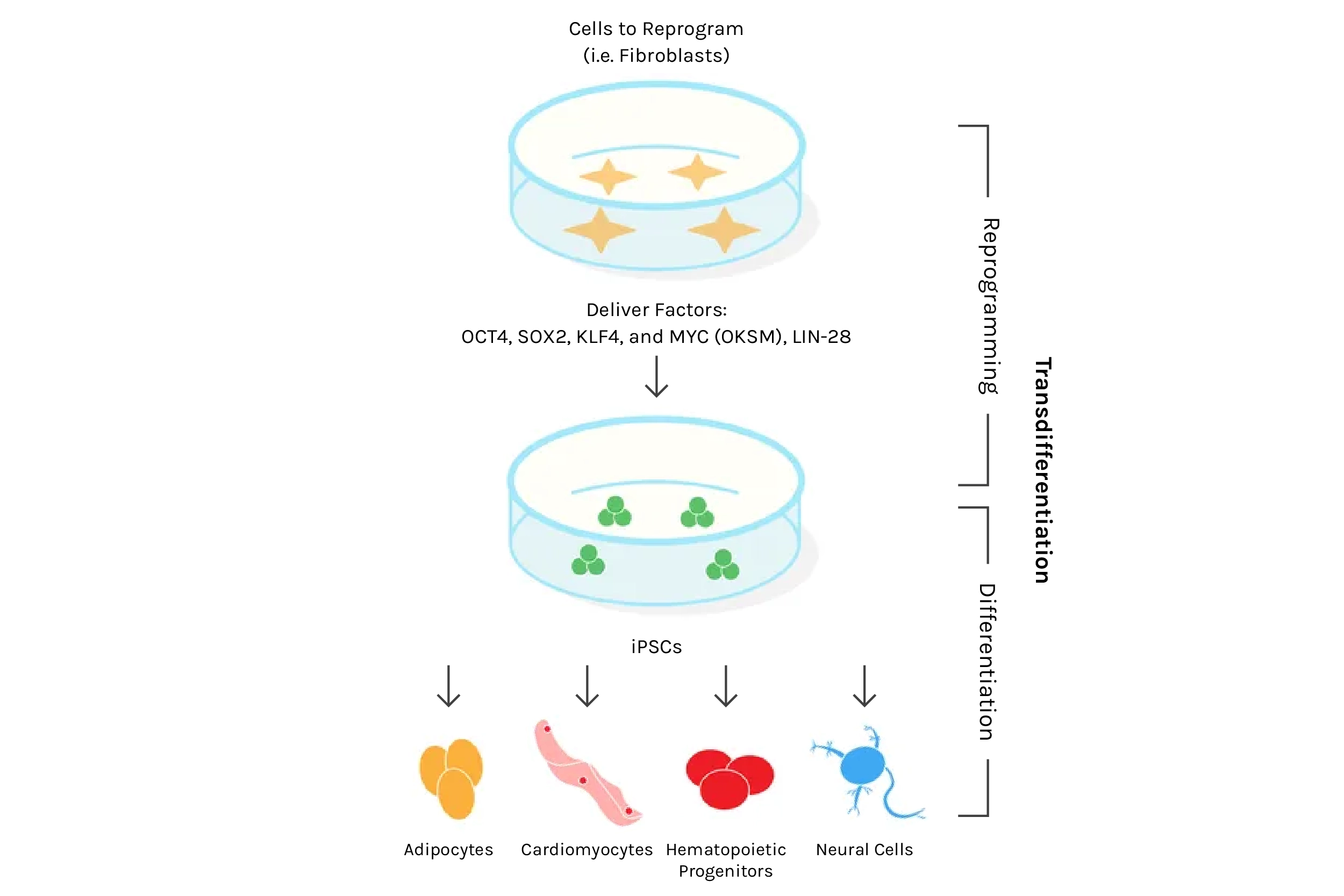
Source: Plasmids for Stem Cell Research
And despite the staggering potential for iPSCs in medicine (more on that next time when we discuss replacing aged organs and cells), only a few recognized the arguably even bigger potential — the possibility of rejuvenating the body’s trillions of cells via reprogramming. With the newfound ability to reprogram cells at will, could we thus rejuvenate any mature cell? Could this be a universal age-reversal therapy? It wasn’t immediately obvious this could work. After all, reprogramming converts mature cells into highly replicative embryonic-like stem cells, the same type known to lead to horrendous tumors composed of different tissues and cell types called teratomas. However, in 2016, researchers tackled this challenge by employing what they termed “partial reprogramming,” a scheme by which the four iPSC genes are turned on and off cyclically every few days. Remarkably, this appeared to induce rejuvenation without the loss of cell identity that leads to teratomas, and the authors reported lifespan extension in a genetic mouse model of premature aging.
This marked a seminal moment in the aging field, triggering an explosion of research and investment, both in academic circles and for-profit biotechnologies companies seeking to capitalize on new therapies for humans. Making headlines in 2021, Altos Labs, replacing the fledgling philanthropic Milky Way Research Foundation, emerged as perhaps the best-funded biotechnology company ever established. Following a flurry of recruiting top talent and renowned figures in reprogramming and the closely associated field of DNA methylation aging clocks, Altos now encompasses over 20 PI-led laboratories across three sites: San Diego, San Francisco, and Cambridge, UK.
While the excitement is perhaps warranted — as reprogramming stems from what is arguably the most fundamental way to elicit cellular age-reversal found in nature — the field remains nascent, with dozens of fundamental questions yet unanswered. What features of aging does reprogramming genuinely reverse? Is that reversal permanent, or will cells revert to their aged state? Are other features of aging missed that make reprogramming moot? How often must one perform reprogramming to maintain youthful function? Can reprogramming ever be deemed safe? Does the technology even exist to perform reprogramming in humans, or to deliver the factors to the right organs and cell types? Do different cell types require different factors? Are there factors superior to the Yamanaka factors? Can we separate rejuvenation from the loss of cell identity?
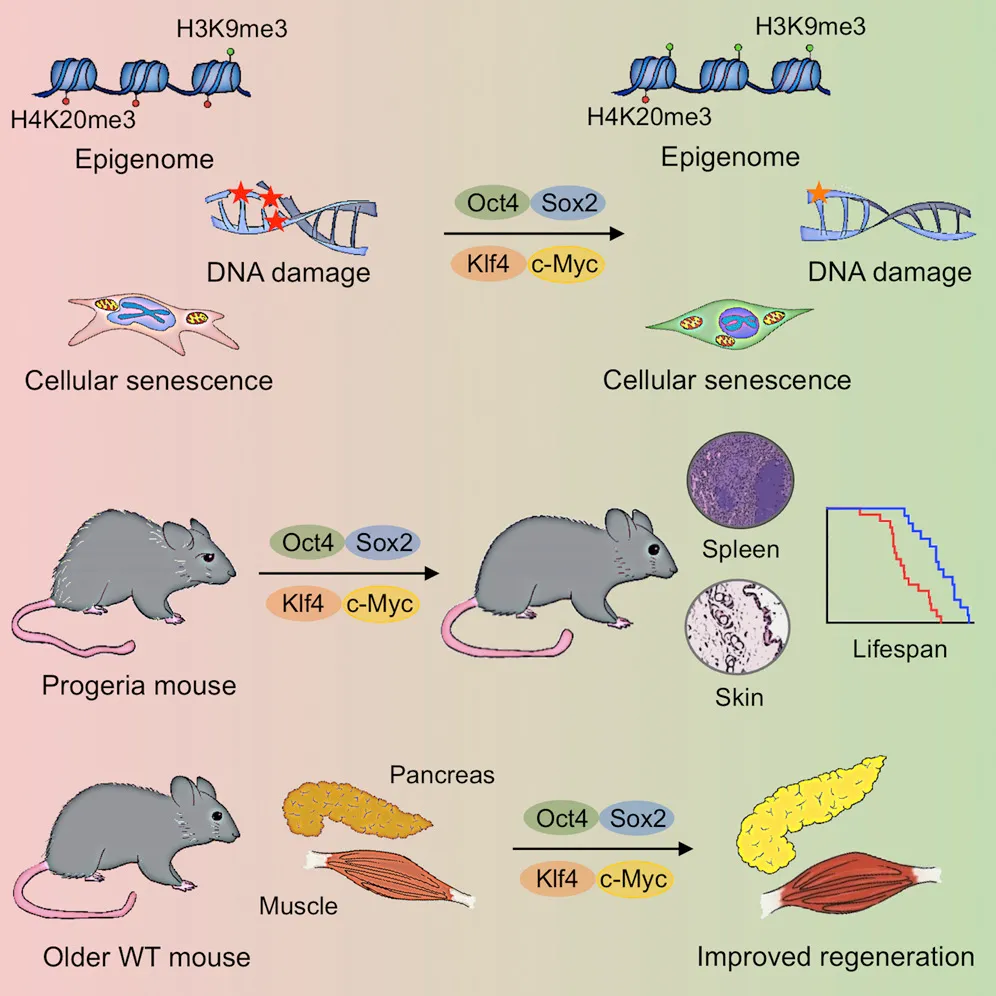
Source: In Vivo Amelioration of Age-Associated Hallmarks by Partial Reprogramming
The sprint for answers is on, with potentially billions of dollars at stake. At the heart of this race is safety — the pursuit for novel reprogramming methods that avoid erasing cellular identity while preserving rejuvenation. Strategies fall into a few categories, the first of which we’ve already visited: cyclic bursts or transient induction of the Yamanaka factors. For example, Turn Biosciences delivers messenger RNA (mRNA) via lipid nanoparticles (like some Covid vaccines) to instruct cells to produce the four Yamanaka factors (and, at Turn, two additional mRNAs for Lin28 and Nanog). But, as mRNAs degrade relatively quickly, the effect is a brief burst of reprogramming.
Others attempt to reduce teratoma risk with a subset of Yamanaka factors, commonly OSK rather than OSKM. These include Life Biosciences, a company advancing research that demonstrated regeneration of the optic nerve with OSK, and Rejuvenate Bio, which recently showed lifespan extension after OSK delivery via adeno-associated viruses (AAVs) to mice of very advanced age.
Several companies are integrating research on established Yamanaka factors with platforms to discover novel factors. YouthBio Therapeutics has undertaken this approach, conducting tests in mouse models of both progeria (an “accelerated aging” genetic disease) and Alzheimer’s by targeting specific cell types like muscle cells and neurons. Others also avoid pan-reprogramming, opting instead to target specific cells. Retro Biosciences, for instance, investigates both novel and Yamakaka factors for reprogramming hepatocytes (the main liver cell type), T cells (an immune cell with many therapeutic applications), and hematopoietic stem cells (the fundamental stem cell that gives rise to all immune cells). NewLimit is among the few companies wholly dedicated to reprogramming, targeting both hepatocytes and T cells using high-throughput screening combined with machine learning (ML) to test and predict countless combinations of novel factors.
In fact, this approach is no longer the exception, but the norm, with many biotech companies combining novel factor screening, ML, and readouts like DNA methylation “aging clocks” to assess reprogramming success and safety. For example, Shift Bioscience has developed an accurate transcriptome clock for single cells based on the abundance of thousands of different mRNAs, with plans to iteratively screen and predict novel reprogramming factors with an ML ‘cell simulation’. Following this theme, the newly launched Junevity similarly utilizes non-Yamanaka factors for reprogramming. Finally, the just-announced Moonwalk Biosciences features a fundamentally different DNA methylation-centric approach, using tools to modify methylation more directly, rather than supplying factors that lead to downstream methylation changes.
DNA methylation, remember, is critical for governing which DNA instructions (genes) are read and thus which proteins the cell produces, which in turn governs cellular function and identity. And methylation is measurable, giving insight not only into the success of reprogramming, but also indicating the “age” of the cell. Since the inception of the first DNA methylation “clocks,” this method has been adapted by dozens of direct-to-consumer companies. Through a blood sample, they claim to determine if an individual is younger or older than their chronological age, with repeated measurements potentially uncovering your “rate” of aging. Some companies pair DNA methylation reports with supplements, exercise regimens, and special diets. However, the true value of this information and whether one can or should act on the results remains somewhat dubious, as blood tests measuring DNA methylation typically mix different cell types, making it unclear if the DNA methylation profile is a result of aging, changes in cell type proportions, or both. Fortunately, research is progressing rapidly, with single-cell DNA methylation clocks on the way.
Despite these considerations, reprogramming and DNA methylation remain hot topics, with the field poised for exponential growth. Whether this represents our best bet for the next generation of medicine remains to be seen. Undoubtedly, reprogramming stands out as one of the few methods capable of eliciting a true reversal of at least some aspects of molecular aging, not just a slowing of progression. By mimicking the immortal germ line — nature’s most robust method of rejuvenation — through targeting the driving hallmark of aging that is epigenetic alterations, reprogramming may indeed hold the potential to significantly improve the consequences of aging at the molecular, functional, and organismal level.
But what if reprogramming falls short? What if some hallmarks of aging aren’t reversed? What if traditional approaches are just too simple for the immense complexity of aging? An increasing number of scientists argue this is likely — that reversing the extremely complex phenomenon of aging is just too great a challenge. So why not avoid this altogether? Why not avoid wasting billions of dollars on drug development and the manipulation of individual molecular targets, and instead replace the aged components altogether? If your cells get old, don’t try to make them young, just replace them! We already do this in the clinic. Organ transplants and immune cell transplants are common. If we could just do this for more of our parts, wouldn’t that be the most efficient way to prevent aging and the horrible diseases it instigates? While the field grapples with ethical and technological hurdles, substantial progress is underway in more ways than you probably imagine, with implications not only for aging but for the thousands relegated to transplant wait lists.
Replacement: When Young at Heart is Just Not Enough
Following our introduction to aging research and foray into reprogramming for rejuvenation, we now turn to replacement, the notion that the best way to tackle aging and remain disease-free may be the most audacious approach of all: systematically replacing our old organs, tissues, and cells with young ones. While this may seem outlandish, in theory, it should work. A kidney transplant here, a new liver there, and voila! Good as new. In practice, of course, the human body is not a machine. Replacing a part isn’t as easy as unscrewing a few bolts. Organ transplants are intricate and major operations, and recipients, if they are lucky enough to find a matching donor in the first place, must take immunosuppressive drugs indefinitely. Not to mention that the parts aren’t readily available anyway — there is a huge shortage of human organ donors with no reliable or scalable alternative source. Oh, and there’s the issue that some organs, like the brain, seemingly defy replacement altogether (though some are trying).
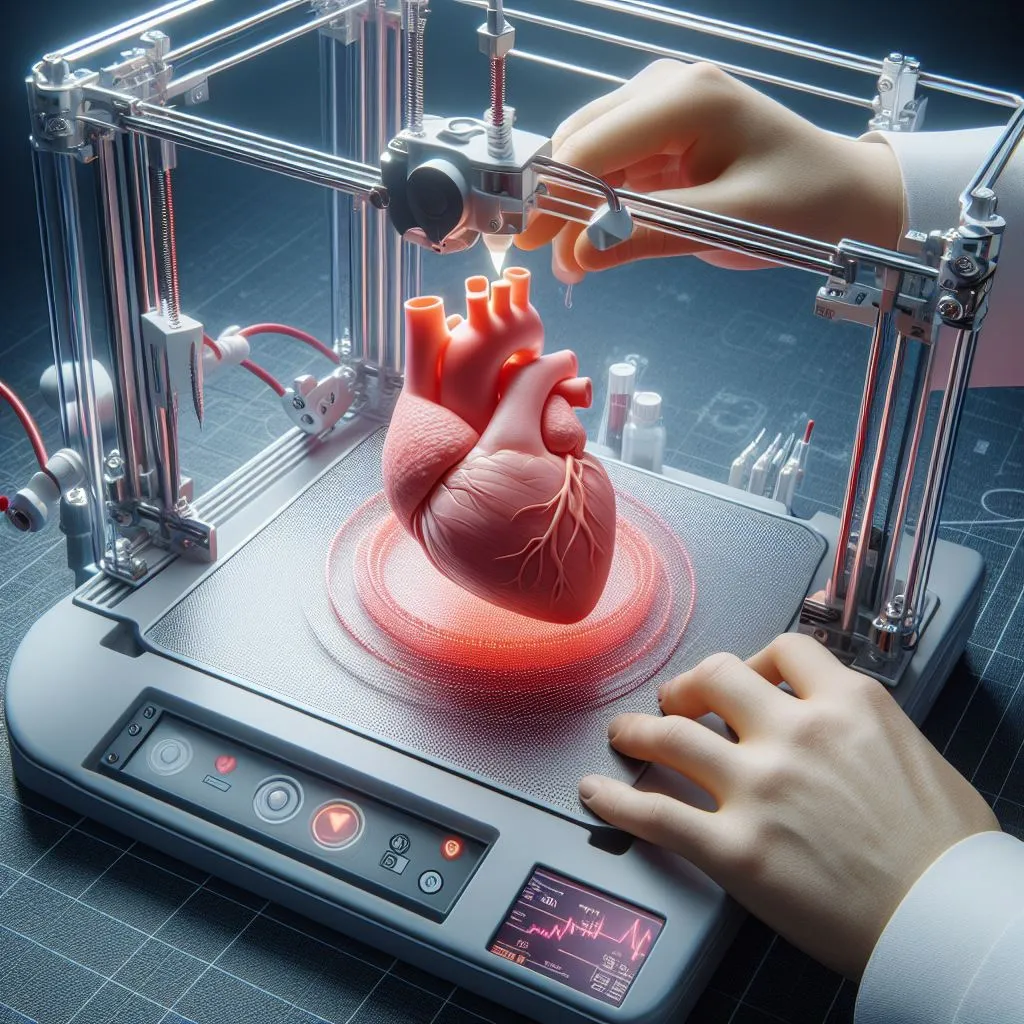
Despite the challenge, the prospect that I might help make replacements viable was, in part, what drew me to aging research. Back in 2010, when I first discovered that scientists seriously researched aging and tissue engineering was hot. The recent discovery of the Yamanaka factors had opened seemingly endless possibilities to create new tissues and organs at will from induced pluripotent stem cells (iPSCs). This concept of “replacement” led me to a lab exploring the intersection of blood — arguably the easiest “part” to replace — and brain rejuvenation. If you can’t replace the brain itself, I thought, would replacing everything else help rejuvenate the brain? After all, our organs depend on each other for proper function, and blood serves as a major communication highway.
One particular technique caught my eye: heterochronic parabiosis — a Frankenstein-esk method involving the surgical attachment of two mice of different ages side by side. Once sewn, skin and blood vessels fuse, establishing a shared circulatory system in which young blood travels into the old mouse, and vice versa. At the time, parabiosis had already generated intriguing results, regenerating capacity in the liver and muscle, and detrimental effects to the brains of young animals. Researchers would also come to observe cognitive benefits in older brains after parabiosis, plus additional benefits across several more major organs. And, experiments involving young plasma (the cell-free liquid portion of blood) injections suggested that the benefits of parabiosis were mediated at least in part by soluble factors.
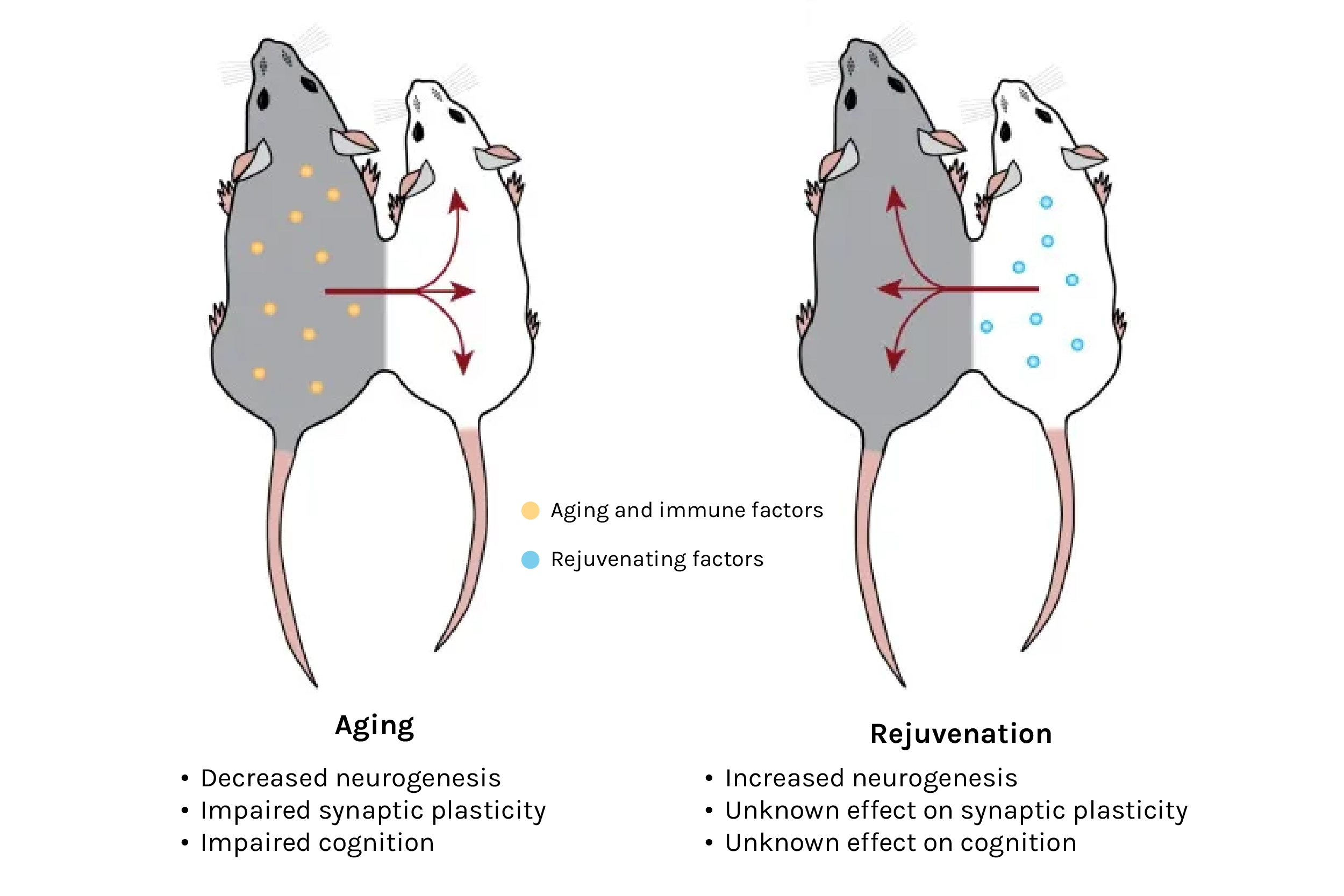
Source: The Circulatory Systemic Environment as a Modulator of Neurogenesis and Brain Aging
While the media tends to sensationalize these findings with comparisons to the fountain of youth, vampires, and “blood boys” (though these are indeed also real), the practical challenges of transfusing young blood for therapeutic purposes are substantial. There’s simply not enough blood to go around. So instead, what if we could identify the blood components responsible for rejuvenation? Could we then mass produce and use those blood factors to prevent aging and disease? A decade ago, many labs had already begun the search, mostly focusing on proteins in plasma. While not the only type of biomolecule in blood — lipids, sugars, small metabolites, and even cell-free DNA can be found — early evidence pointed to proteins, spawned several companies like Alkahest hoping to treat dementia with particular plasma protein fractions (that is, proteins within a specific size range) found in young blood, and Elevian, applying a particular protein called GDF11 to stroke. Others continue to explore new secreted factors, like those that modulate immune responses at Immunis Biomedical, and those derived from stem cells at Juvena Therapeutics. Newer to the area is Retro Biosciences, reportedly focusing on plasmapheresis — a common method used to remove and replace a patient’s plasma.
But what about the source of these proteins? Where were they coming from? Could we identify the old organ that stops producing beneficial factors and stimulate more production, or replace that old organ with a young one to rejuvenate its production? These were the questions I sought to answer when I joined the field. To begin, I collected all major mouse organs across the lifespan and then performed RNA-sequencing, a method that measures the “expression” of all 20,000+ genes in a sample. Remember that genes are specific DNA instructions for how to make proteins. Each different gene makes a different protein. But there is an intermediate step — first, each gene is transcribed into a message, an mRNA molecule, the type that is read with RNA-sequencing. Each gene can be transcribed many times, such that you may have 5,000 mRNA copies of gene A, 400 of gene 4, and so on. These mRNA messages are then read by other cellular machinery that creates a specific protein with a specific role in, on, or outside of the cell, in a process called translation. So, the more mRNAs for a given gene, the more proteins of that gene are synthesized.
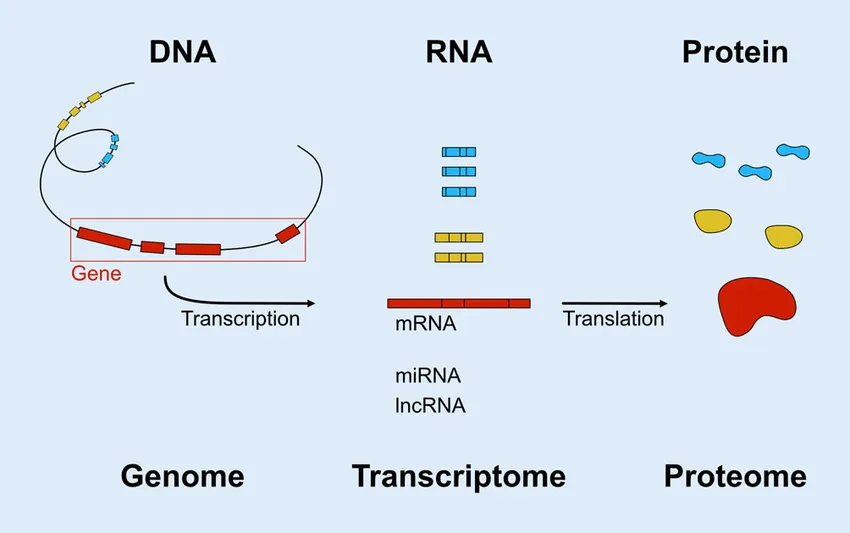
Adapted from: Web-based Gene Expression Analysis-paving the Way to Decode Healthy and Diseased Ocular Tissue
While not a perfect correlate to protein abundance (sometimes mRNA levels and protein levels vastly differ), this expression does often predict the amount of the corresponding protein in the sample, and because proteins have known roles in the cell, one can infer what has changed with age. In this way, early experiments indicated immune-related changes were among the largest contributors to aging throughout the body. Although my research took a different direction, other members of the lab pursued a similar concept in humans, leading to the newly founded Teal Omics. The goal: predict aging of internal organs using minimally invasive blood draws.
These early discoveries of immune changes with age sparked an idea. Rather than replace each component of blood, why not replace the source of blood itself: the bone marrow? Bone marrow not only generates our red blood cells and platelets, but it also produces white blood cells that guard against infection. As immune function diminishes with age, vaccines lose efficacy and the elderly become drastically more susceptible to communicable diseases. There’s no clearer example in recent history than COVID-19, but this vulnerability to infection underlies more deaths than commonly appreciated. For instance, falls lead to broken hips and surgery, which can result in infection that leads to death. If we could just replace the bone marrow, we could rejuvenate the body’s entire immune AND communication system.
However, akin to whole organ transplants, bone marrow transplants are reserved for life-and-death situations. This necessity arises from the highly toxic conditioning regimens employed to eradicate the recipient’s native bone marrow, creating space for the transplanted cells. These treatments, while assisting to destroy the cancer, subject patients to extreme discomfort and leave them without an immune system, subjecting them to excessive risk of infection for months. In an experimental setting, the situation is similar: whole-body irradiation is standard for bone marrow transplants in mice. Unfortunately, this makes experiments transplanting young bone marrow to aged mice a bit difficult to interpret, though even so, several labs have reported benefits to multiple organ systems, including the brain, and younger human donors correlate with improved survival in patients with at least some types of blood cancer (unpublished data).
Actually, my optimism about heterochronic bone marrow transplantation arose from work of a neighboring lab that developed a novel method of bypassing harsh conditioning regimens. Instead, these researchers specifically targeted and eliminated hematopoietic stem cells (HSCs) — the cells from which the entire immune system arises. They would then transplant not whole bone marrow, but just HSCs, which can be collected from blood after coaxing them to leave the marrow (this means donating “marrow” is as easy as donating blood — sign up at Be The Match).
Not only is this research critical for blood cancer patients, but unlocking safe conditioning regimens would open the door for HSC transplantation to address various maladies, if not aging itself. After all, immune cells are systemic, traversing and living throughout the entire body, not only to search for pathogens but helping to maintain normal organ function. Unsurprisingly, the immune system and inflammation are either the direct causes of or associated with nearly every disease. Replacing this every so often could have untold benefits for maintaining health and preventing chronic diseases from emerging in the first place. The new conditioning regimen specific for hematopoietic stem cells is now undergoing development at Jasper Therapeutics, and could help make this a reality. And, though I failed to achieve sufficient engraftment of young HSCs in my experiments, others succeeded and demonstrated lifespan extension in mice. Currently, the LEV Foundation is attempting to reproduce these results, with lifespan experiments underway.
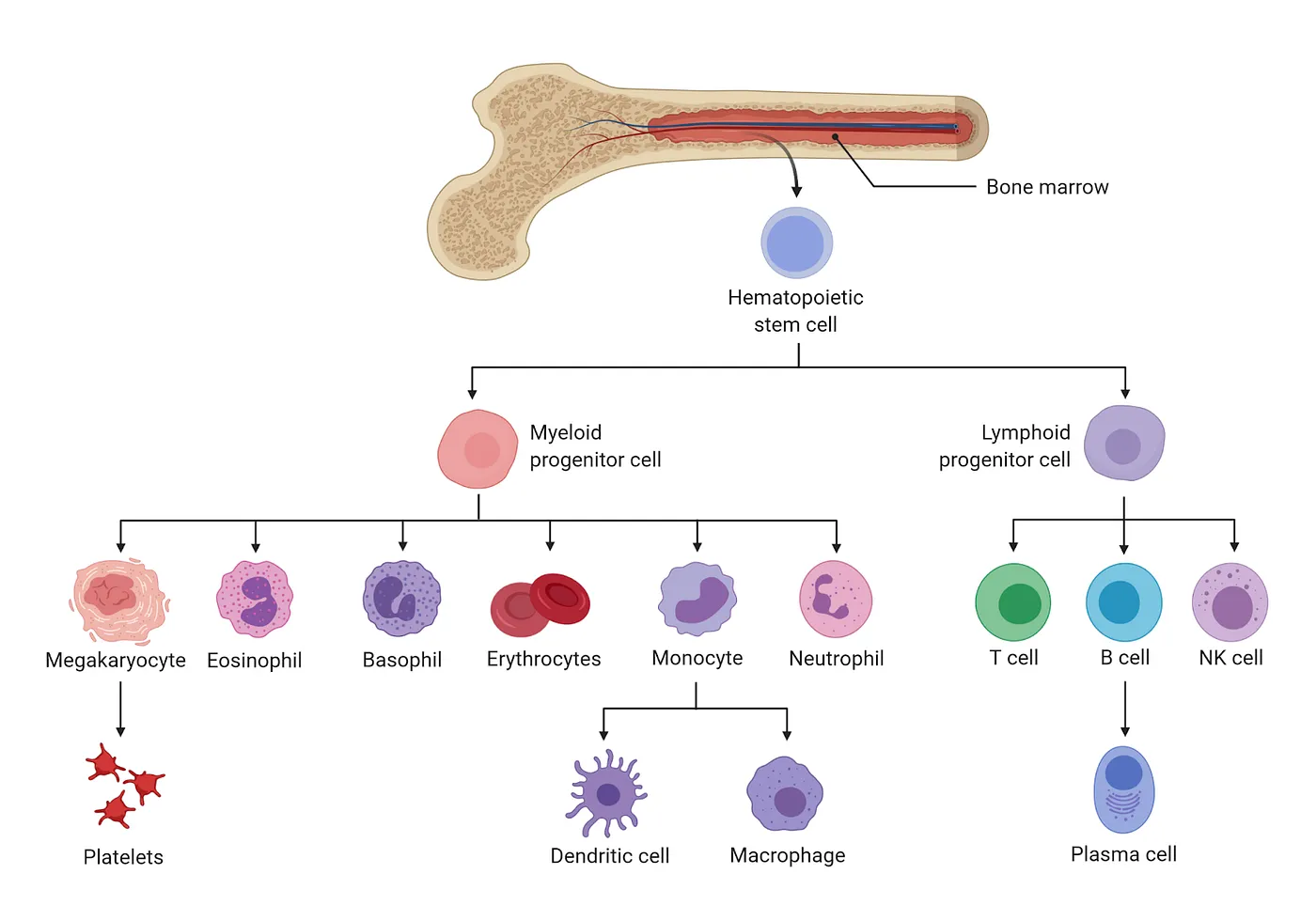
Source: The Cardiovascular Research Institute at Mount Sinai
The immune system is so central to aging and disease that scientists are attempting a variety of rejuvenation approaches. For example, ImmuneAge Bio aims to reboot the immune system by rejuvenating and expanding HSCs ex vivo (outside of the body) before reinfusing them into patients. Mogling Bio utilizes a Cdc42 inhibitor which appears to restore order to the inner scaffolding of old cells, a treatment that extended mouse lifespan even when administered transiently after the mice had already reached old age. Minova Therapeutics has developed a method of transplanting placenta-derived mitochondria into HSCs, both as a treatment for rare mitochondrial diseases and also blood cancers. The potential transfer of those healthy mitochondria from the injected HSCs to other cells in the body means the therapeutic benefit could extend beyond the immune system. And of course, there are companies reprogramming HSCs like Retro Biosciences. In addition, both Retro and NewLimit, target T cells — specific immune cells used in certain cancer therapies.
Others also work on tools that may aid in the pursuit of replacement. Ossium Health is establishing a bone marrow bank while exploring ways to reduce transplant rejection, and they also provide bone matrix grafts from organ donors. Gamida Cell’s nicotinamide-based Omisirge may help with recovery from transplantation by accelerating neutrophil recovery, reducing infection risk. In addition, Videregen is working to regenerate the thymus, a small organ that sits above the heart where T cell development takes place. With age, the thymus undergoes drastic atrophy, practically disappearing by middle age. Thymmune Therapeutics — making headlines recently after receiving $37M from the Advanced Research Projects Agency for Health (ARPA-H) — also works on this historically neglected area.
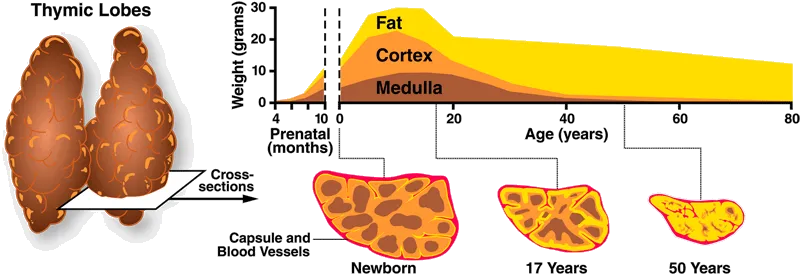
Source: Immune System Immunity
One particularly exciting concept, while not targeting aging per se, is the idea of manufacturing blood components, like red blood cells (RBCs), outside of the body, thus eliminating the need for human donors. Working to manufacture universal-donor RBCs at industrial scale, RedC Biotech could help tackle a huge unmet clinical need. Others are performing similar work, though Scarlet Therapeutics intends to use RBCs as carriers for therapeutics.
With the ease of access to blood and its components, efforts in replacement and rejuvenation are expanding rapidly. But beyond this, the idea of replacement has taken off as well, and perhaps for good reason. A growing number of well-respected researchers believe that our best bet for keeping people healthy longer is to bypass traditional approaches that attempt to manipulate the immense complexity of molecular biology, and instead replace worn organs with young ones. The argument is that there are just so many processes that go wrong, and so many types of cellular and molecular damage to reverse, that it would take immense technological advances and concurrent therapies to fix them all. Generating and transplanting a fresh organ, many would argue, is actually far easier to accomplish.
Though voicing this opinion seriously remains somewhat new, the transplantation field is over half a century old. In fact, one of its pioneers, Roy Calne, recently passed away. But clearly, human donors are insufficient even to meet our current needs. So how could we imagine a future where everyone receives a new liver, and kidney, and heart?
One clever approach, taken by LyGenesis, could help alleviate the organ shortage by enabling each liver donation to benefit multiple patients. They accomplish this by isolating hepatocytes — the central cell type of liver — from the donated tissue and then injecting those into the patient’s lymph node with an endoscope. The lymph node provides an environment that naturally promotes cell growth, and the donated cells expand to form a mini-liver. For patients with end-stage liver disease, this could be lifesaving. Satellite Bio employs a similar concept by implanting satellite adaptive tissues (SATs), palm-sized disks of cells that become vascularized once inside the body. Another unique approach is that taken by Morphoceuticals, who champion instigating innate regeneration, arguing that the body already contains instructions for how to generate organs and limbs — it just needs the right signals. Utilizing a combination of factors delivered via a wearable bioreactor, they have successfully regenerated frog limbs.
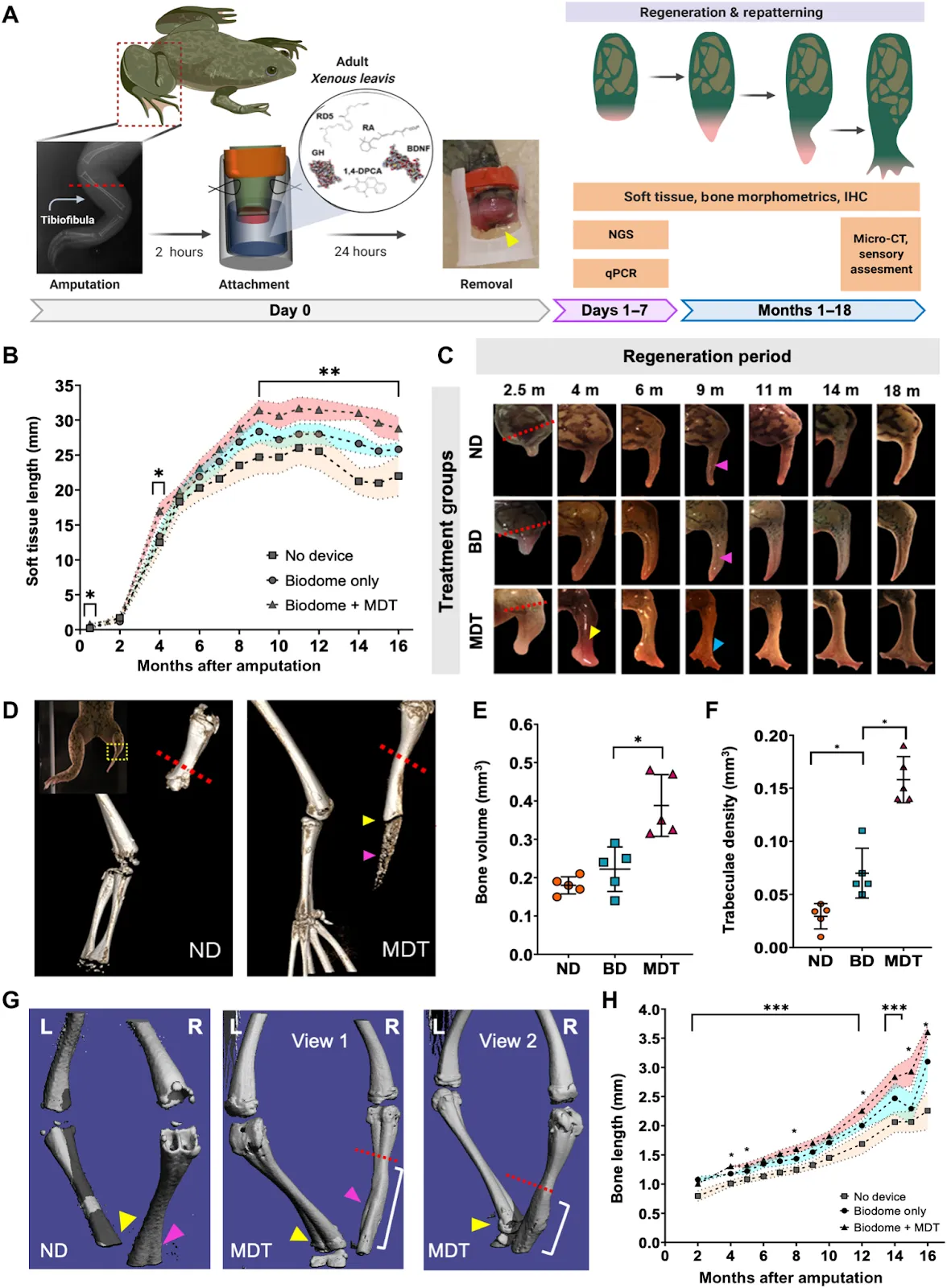
Others take the engineering perspective, like Iviva Medical with 3D-printed cell-free microfluidic scaffolds seeded with kidney cells. With the goal of eliminating the need for dialysis, the similar Trestle Biotherapeutics generates implantable kidney tissues. And indeed, implantables receive much focus, as these pair therapeutic benefits with the ease of manufacturing. For example, Dimension Inx creates implantable bio-ink seeded bone and ovary scaffolds, and Humacyte generates blood vessels by seeding an absorbable scaffold with cells that subsequently deposit their own scaffold. Once the cells are removed, what remains is an acellular vessel useful for vascular disease or trauma without fear of rejection. Similarly, Verigraft decellularizes donated veins, arteries, valves, and nerves before reseeding the resulting scaffolds with a patient’s own cells.
Another major category of investigation is xenotransplantation — transplanting organs from non-human organisms, typically pigs due to their similar size to humans. United Therapeutics, through its subsidiary Revivicor and recent acquisition of Miromatrix, is advancing programs for a number of major organs, including decellularized pig organs reseeded with human cells to enable rejection-free transplantation. Revivicor uses a different approach to tackle this goal, genetically engineering porcine cells to be resistant to immune rejection. After combining these cells with pig egg cells in a technique called somatic cell nuclear transfer, all cells of the resulting embryo inherit the engineered genome. Once an adult, the organs are suitable for xenotransplantation. This research appears full steam ahead as Revivicor expands with a $100 million pig-organ facility. And they are not alone. Qihan Biotech similarly edits pig genomes to inactivate the endogenous retroviruses that pose a risk to humans, and Makana Therapeutics utilizes a triple knock-out to rid the pig of three specific cell-surface molecules that provoke immune rejection. eGenesis pursues similar goals, successfully performing 69 gene edits on kidneys transplanted into monkeys. When combined with immunosuppressants, these kidneys survived for up to two years.
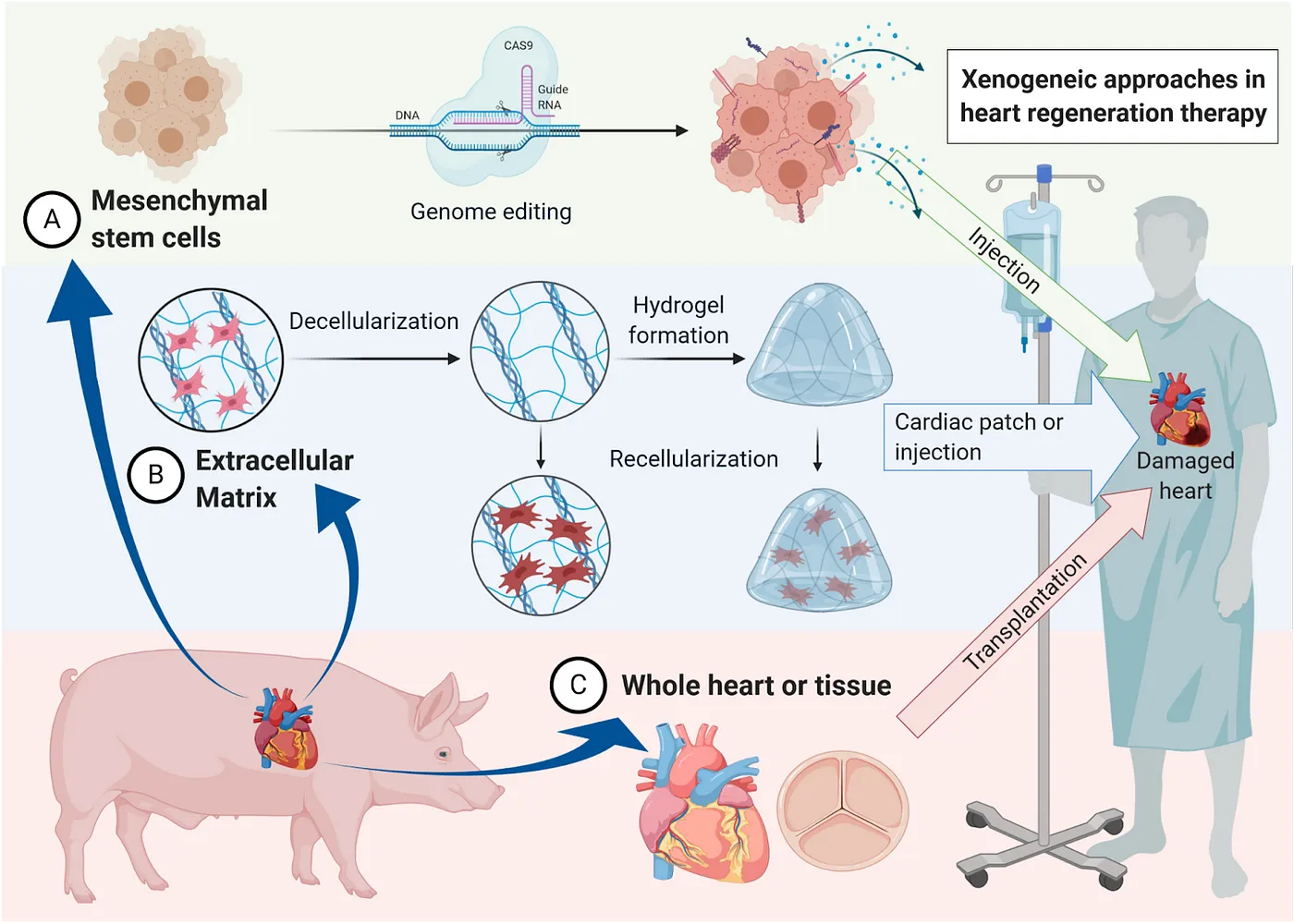
While xenotransplantation remains hot, driven by the advances in Nobel prize-winning CRISPR gene editing technologies, room for improvement remains, as current gene-edited xenotransplants still appear to elicit rejection in humans. Alternatively, the oft-overlooked gut microbiome may provide benefits without the hassle of immune rejection — some evidence suggests benefits to old animals receiving a young fecal microbiota transplantation (FMT), including lifespan extension in fish, and improvements in the gut, brain, and eye. And, FMT is already used in the clinic to treat recurrent C. difficile infections, though here too, opportunities for progress abound.
If there is one organ that cannot be transplanted, it is the brain. Although other organs are complex to replace for other reasons — just think of our 600+ muscles or our two square meters of skin — the brain is you. You can’t just get a new one like you can a new kidney. But perhaps, some would argue, it might just be possible to replace the brain little by little, leaving you intact all the while. A number of companies pursue cell therapies — using stem cells to generate brain cell types for injection to treat neurodegenerative diseases. A few pursue more unique methods, like NeuExcel’s in vivo conversion of astrocytes to neurons. In theory, such a method could supply new neurons to the aging brain to reverse neurodegeneration. And then there is Glionics, a company aspiring to microglia replacement in humans. This technique is based on experiments demonstrating the complete ablation of microglia in the adult mouse brain followed by reseeding the brain with young microglia, which soon populate regions far from their site of administration.
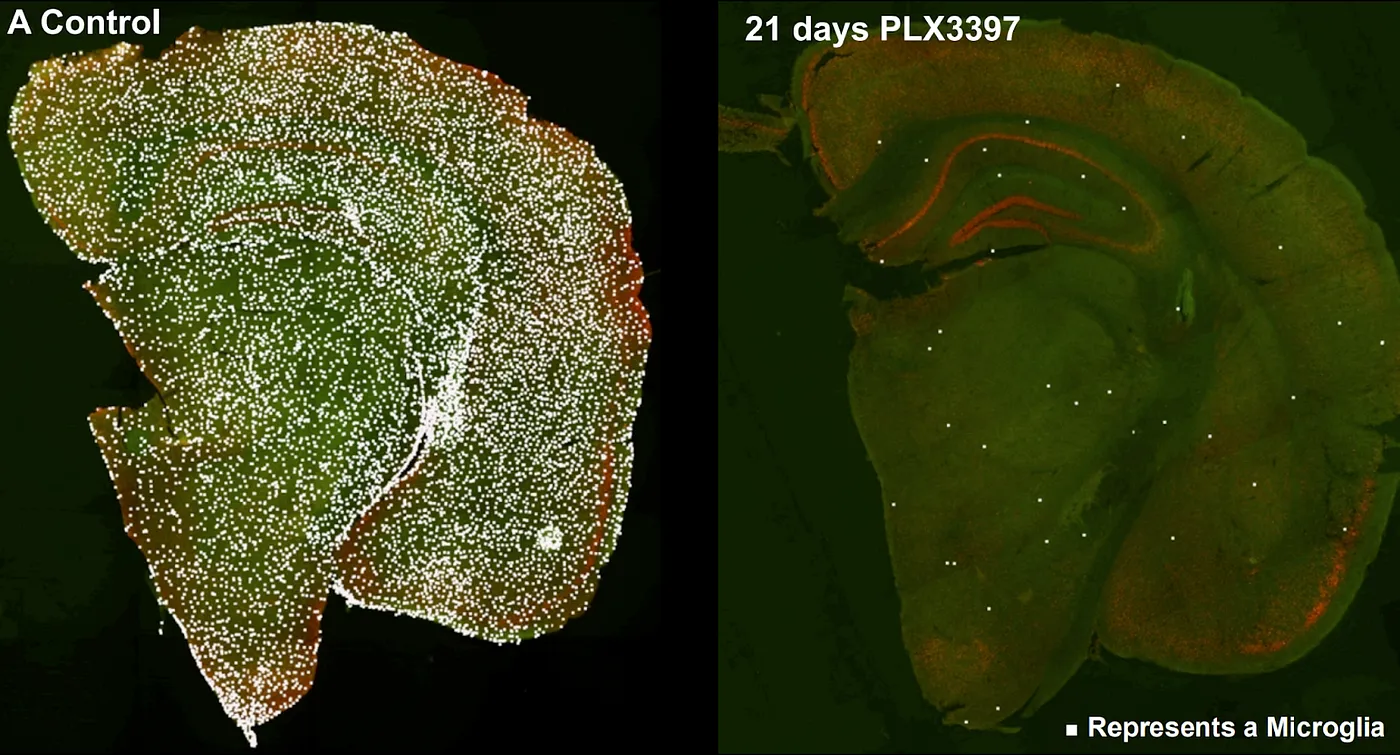
Ablation of microglia with a CSF1R inhibitor, PLX3397, in the mouse brain. Source: Colony-stimulating Factor 1 Receptor Signaling is Necessary for Microglia Viability, Unmasking a Microglia Progenitor Cell in the Adult Brain
And finally, there are the more speculative approaches that make global headlines — wild experiments transplanting an entire head, for example. This is, according to Wikipedia, distinct from brain transplantation, or looked at from the reverse perspective, whole-body transplantation. These experiments face not only extremely challenging technical hurdles, like how to attach nerves and blood vessels and prevent immune rejection, but serious ethical considerations, should technology develop enough to make this a reality.
But, many bet on just this future, cryopreserving their heads in the hopes that our future society has the tools to reanimate them with new, young bodies. I know what your impression must be, but cryopreservation for medical purposes is serious business. Methods to better preserve donor organs would have a huge impact on the thousands relegated to waitlists, preventing waste, and granting critical flexibility to donate across long distances, which is often a huge logistical challenge if not an impossibility when live organs are required. Lately, several cryopreservation efforts have been launched by aging researchers, including Oxford Cryotechnology and Lorentz Bio. While some might argue that cryopreservation is Plan B, more people seem to be converting to cryo as Plan A.
But not everyone. To close, we visit Renewal Bio, whose work, if successful, could potentially supplant at least some of the above replacement approaches. Renewal is at the forefront of ex utero human embryogenesis. While it is tempting to theorize this could lead to personalized clones that could supply patient-specific organs, it’s important to remember that 1) the technology is not in place to advance embryos this far, and 2) there are stringent rules limiting the duration of embryonic development. In the future, it may be that such development is allowed to progress (or not, depending on the country or state), so long as brain development is fully prevented — something possible already in mice, for example, by turning off a specific gene called Lim1. Even considering these limitations, within only a few weeks, multiple cell types of huge therapeutic potential arise, including eggs to treat infertility, pancreatic beta cells for diabetes, and hematopoietic stem cells for cancer and anemia. And, using iPSCs, all of these could be patient-specific! Why go to the trouble and complexity of engineered tissues and pig organs when you can generate therapeutics directly from human cells?
From replacing blood to bone marrow, to solid organs and our brains themselves, the borders between reality and science fiction are more blurred than ever. Each replacement approach above has its own merits and drawbacks in relation to traditional approaches like drug development. But ask yourself, do those pursuing replacement as a solution to aging and disease have a point? Is moving beyond the hallmarks of aging, by solving them all at once with young organs, actually the most promising way to stave off aging and help prevent dementia, diabetes, heart disease, and cancer? Only time will tell — hopefully for us, not too much of it.